The changing state of porous materials
Porous materials contain regions of empty space into which guest molecules can be selectively adsorbed and sometimes chemically transformed. This has made them useful in both industrial and domestic applications, ranging from gas separation, energy storage and ion exchange to heterogeneous catalysis and green chemistry. Porous materials are often ordered (crystalline) solids. Order—or uniformity—is frequently held to be advantageous, or even pivotal, to our ability to engineer useful properties in a rational way. Here we highlight the growing evidence that topological disorder can be useful in creating alternative properties in porous materials. In particular, we highlight here several concepts for the creation of novel porous liquids, rationalize routes to porous glasses and provide perspectives on applications for porous liquids and glasses.
This is a preview of subscription content, access via your institution
Access options
Access Nature and 54 other Nature Portfolio journals
Get Nature+, our best-value online-access subscription
cancel any time
Subscribe to this journal
Receive 12 print issues and online access
265,23 € per year
only 22,10 € per issue
Buy this article
- Purchase on SpringerLink
- Instant access to full article PDF
Prices may be subject to local taxes which are calculated during checkout


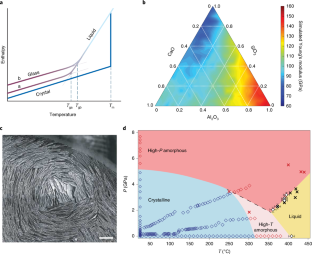
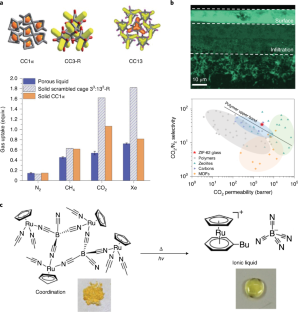
Similar content being viewed by others
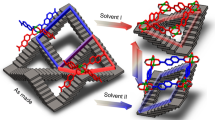
Adaptive response of a metal–organic framework through reversible disorder–disorder transitions
Article 27 May 2021
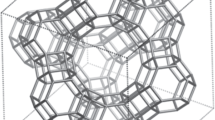
Atoms and the void: modular construction of ordered porous solids
Article Open access 16 September 2020
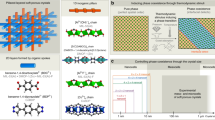
Unraveling the thermodynamic criteria for size-dependent spontaneous phase separation in soft porous crystals
Article Open access 24 October 2019
References
- Wright, P. A. The Chemistry of Microporous Framework Solids (Royal Society of Chemistry, 2008).
- Davis, M. E. Ordered porous materials for emerging applications. Nature417, 813–821 (2002). ArticleCASGoogle Scholar
- Čejka, J., Morris, R. E. & Nachtigall, P. Zeolites in Catalysis: Properties and Applications (Catalysis Series Vol. 28, Royal Society of Chemistry, 2017).
- Furukawa, H., Cordova, K. E., O’Keeffe, M. & Yaghi, O. M. The chemistry and applications of metal–organic frameworks. Science341, 974–986 (2013). ArticleCASGoogle Scholar
- Barrer, R. M. Syntheses and reactions of mordenite. J. Chem. Soc. https://doi.org/10.1039/JR9480002158 (1948).
- Giannakoudakis, D. A. & Bandosz, T. Detoxification of Chemical Warfare Agents: from WWI to Multifunctional Nanocomposite Approaches (Springer, 2018).
- Patrick, W. Silica gel and process of making same. US patent US1,297,724A (1919).
- Yanagisawa, T., Shimizu, T. & Kuroda, K. The preparation of alkyltriinethylaininonium–kaneinite complexes and their conversion to microporous materials. Bull. Chem. Soc. Jpn63, 988–992 (1990). ArticleCASGoogle Scholar
- Budd, P. M. et al. Polymers of intrinsic microporosity (PIMs): robust, solution-processable, organic nanoporous materials. Chem. Commun.2, 230–231 (2004). ArticleCASGoogle Scholar
- Ben, T. et al. Targeted synthesis of a porous aromatic framework with high stability and exceptionally high surface area. Angew. Chem. Int. Ed.48, 9457–9460 (2009). ArticleCASGoogle Scholar
- Cote, A. P. et al. Porous, crystalline, covalent organic frameworks. Science310, 1166–1170 (2005). ArticleCASGoogle Scholar
- Williams, K. A., Boydston, A. J. & Bielawski, C. W. Main-chain organometallic polymers: synthetic strategies, applications, and perspectives. Chem. Soc. Rev.36, 729–744 (2007). ArticleCASGoogle Scholar
- Honicke, I. M. et al. Balancing mechanical stability and ultrahigh porosity in crystalline framework materials. Angew. Chem. Int. Ed.57, 13780–13783 (2018). ArticleCASGoogle Scholar
- Cooper, A. I. Porous molecular solids and liquids. ACS Cent. Sci.3, 544–553 (2017). ArticleCASGoogle Scholar
- Ahmad, N., Younus, H. A., Chughtai, A. H. & Verpoort, F. Metal–organic molecular cages: applications of biochemical implications. Chem. Soc. Rev.44, 9–25 (2015). ArticleCASGoogle Scholar
- Carne-Sanchez, A. et al. Self-assembly of metal–organic polyhedra into supramolecular polymers with intrinsic microporosity. Nat. Commun.9, 2506 (2018). ArticleCASGoogle Scholar
- Kitagawa, S., Kitaura, R. & Noro, S. Functional porous coordination polymers. Angew. Chem. Int. Ed.43, 2334–2375 (2004). ArticleCASGoogle Scholar
- Colon, Y. J. & Snurr, R. Q. High-throughput computational screening of metal–organic frameworks. Chem. Soc. Rev.43, 5735–5749 (2014). ArticleCASGoogle Scholar
- Simonov, A. & Goodwin, A. L. Designing disorder into crystalline materials. Nat. Rev. Chem. 4, 657–673 (2020).
- Abednatanzi, S. et al. Mixed-metal metal–organic frameworks. Chem. Soc. Rev.48, 2535–2565 (2019). ArticleCASGoogle Scholar
- Besara, T. et al. Mechanism of the order–disorder phase transition, and glassy behavior in the metal–organic framework [(CH3)2NH2]Zn(HCOO)3. Proc. Natl Acad. Sci. USA108, 6828–6832 (2011). ArticleGoogle Scholar
- Sircar, S. & Golden, T. C. Purification of hydrogen by pressure swing adsorption. Sep. Sci. Technol.35, 667–687 (2000). ArticleCASGoogle Scholar
- Darunte, L. A., Oetomo, A. D., Walton, K. S., Sholl, D. S. & Jones, C. W. Direct air capture of CO2 using amine functionalized MIL-101(Cr). ACS Sustain. Chem. Eng.4, 5761–5768 (2016). ArticleCASGoogle Scholar
- Rochelle, G. T. Amine scrubbing for CO2 capture. Science325, 1652–1654 (2009). ArticleCASGoogle Scholar
- O’Reilly, N., Giri, N. & James, S. L. Porous liquids.Chem. Eur. J.13, 3020–3025 (2007). ArticleCASGoogle Scholar
- Zhang, J. S. et al. Porous liquids: a promising class of media for gas separation. Angew. Chem. Int. Ed.54, 932–936 (2015). ArticleCASGoogle Scholar
- Melaugh, G., Giri, N., Davidson, C. E., James, S. L. & Del Popolo, M. G. Designing and understanding permanent microporosity in liquids. Phys. Chem. Chem. Phys.16, 9422–9431 (2014). ArticleCASGoogle Scholar
- Jie, K. C. et al. Transforming porous organic cages into porous ionic liquids via a supramolecular complexation strategy. Angew. Chem. Int. Ed.59, 2268–2272 (2020). ArticleCASGoogle Scholar
- Ma, L. et al. Coordination cages as permanently porous ionic liquids. Nat. Chem.12, 270–275 (2020). ArticleCASGoogle Scholar
- Akutagawa, T. et al. Nanoscale assemblies of gigantic molecular -rings: (dimethyldioctadecylammonium)20[Mo154O462H8(H2O)70]. Langmuir24, 231–238 (2008). ArticleCASGoogle Scholar
- Pierotti, R. A scaled particle theory of aqueous and nonaqueous solutions. Chem. Rev.76, 717–726 (1976). ArticleCASGoogle Scholar
- Kearsey, R. J., Alston, B., Briggs, M. E., Greenaway, R. L. & Cooper, A. I. Accelerated robotic discovery of type II porous liquids. Chem. Sci.10, 9454–9465 (2019). ArticleCASGoogle Scholar
- Moro, S., Parneix, C., Cabane, B., Sanson, N. & de Lacaillerie, J. B. D. Hydrophobization of silica nanoparticles in water: nanostructure and response to drying stress. Langmuir33, 4709–4719 (2017). ArticleCASGoogle Scholar
- Liu, H. et al. A hybrid absorption–adsorption method to efficiently capture carbon. Nat. Commun.5, 4813 (2014). ArticleCASGoogle Scholar
- Shan, W. et al. New class of type III porous liquids: a promising platform for rational adjustment of gas sorption behavior. ACS Appl. Mater. Interfaces10, 32–36 (2018). ArticleCASGoogle Scholar
- Tian, Y. Q. et al. The silica-like extended polymorphism of cobalt( ii ) imidazolate three-dimensional frameworks: X-ray single-crystal structures and magnetic properties. Chem. Eur. J.9, 5673–5685 (2003). ArticleCASGoogle Scholar
- Gaillac, R. et al. Liquid metal–organic frameworks. Nat. Mater.16, 1149–1154 (2017). ArticleCASGoogle Scholar
- Ediger, M. D., Angell, C. A. & Nagel, S. R. Supercooled liquids and glasses. J. Phys. Chem.100, 13200–13212 (1996). ArticleCASGoogle Scholar
- Giri, N. et al. Alkylated organic cages: from porous crystals to neat liquids. Chem. Sci.3, 2153–2157 (2012). ArticleCASGoogle Scholar
- Hancock, B. C. & Zografi, G. The relationship between the glass-transition temperature and the water-content of amorphous pharmaceutical solids. Pharm. Res.11, 471–477 (1994). ArticleCASGoogle Scholar
- Angell, C. A. Liquid fragility and the glass transition in water and aqueous solutions. Chem. Rev.102, 2627–2649 (2002). ArticleCASGoogle Scholar
- Sare, E. J. & Angell, C. A. Glass-forming composition regions and glass transition temperature in nonaqueous electrolyte solutions. J. Solut. Chem.2, 53–57 (1973). ArticleCASGoogle Scholar
- Yang, K. et al. Predicting the Young’s modulus of silicate glasses using high-throughput molecular dynamics simulations and machine learning. Sci. Rep.9, 8739 (2019). ArticleCASGoogle Scholar
- Lasalle, A., Guizard, C., Maire, E., Adrien, J. & Deville, S. Particle redistribution and structural defect development during ice templating. Acta Mater.60, 4594–4603 (2012). ArticleCASGoogle Scholar
- Zhang, H. F. et al. Aligned two- and three-dimensional structures by directional freezing of polymers and nanoparticles. Nat. Mater.4, 787–793 (2005). ArticleCASGoogle Scholar
- Deville, S., Saiz, E., Nalla, R. K. & Tomsia, A. P. Freezing as a path to build complex composites. Science311, 515–518 (2006). ArticleCASGoogle Scholar
- McMillan, P. F. Polyamorphic transformations in liquids and glasses. J. Mater. Chem.14, 1506–1512 (2004). ArticleCASGoogle Scholar
- McMillan, P. F., Greaves, G. N., Wilson, M., Wilding, M. C. & Daisenberger, D. in Liquid Polymorphism (ed. Stanley, H. E.) 309–353 (Advances in Chemical Physics Vol. 152, Wiley, 2013).
- Ha, A., Cohen, I., Zhao, X. L., Lee, M. & Kivelson, D. Supercooled liquids and polyamorphism. J. Phys. Chem.100, 1–4 (1996). ArticleCASGoogle Scholar
- Sporer, J. The Linde Solinox process: gypsum-free flue-gas desulphurization. Gas Sep. Purif.6, 133–140 (1992). ArticleCASGoogle Scholar
- Sholl, D. S. & Lively, R. P. Seven chemical separations to change the world. Nature532, 435–437 (2016). ArticleGoogle Scholar
- Greenaway, R. L. et al. Understanding gas capacity, guest selectivity, and diffusion in porous liquids. Chem. Sci.8, 2640–2651 (2017). ArticleCASGoogle Scholar
- Cahir, J. et al. Type 3 porous liquids based on non-ionic liquid phases—a broad and tailorable platform of selective, fluid gas sorbents. Chem. Sci.11, 2077–2084 (2020). ArticleCASGoogle Scholar
- Fu, Y. et al. Ultra-thin enzymatic liquid membrane for CO2 separation and capture. Nat. Commun.9, 990 (2018). ArticleCASGoogle Scholar
- Wang, Y. H. et al. A MOF glass membrane for gas separation. Angew. Chem. Int. Ed.59, 4365–4369 (2020). ArticleCASGoogle Scholar
- Whipple, D. T. & Kenis, P. J. A. Prospects of CO2 utilization via direct heterogeneous electrochemical reduction. J. Phys. Chem. Lett.1, 3451–3458 (2010). ArticleCASGoogle Scholar
- Kunene, T., Atifi, A. & Rosenthal, J. Selective CO2 reduction over Rose’s metal in the presence of an imidazolium ionic liquid electrolyte. ACS Appl. Energy Mater.3, 4193–4200 (2020). ArticleCASGoogle Scholar
- Wuttig, M. & Yamada, N. Phase-change materials for rewriteable data storage. Nat. Mater.6, 824–832 (2007). ArticleCASGoogle Scholar
- Funasako, Y., Mori, S. & Mochida, T. Reversible transformation between ionic liquids and coordination polymers by application of light and heat. Chem. Commun.52, 6277–6279 (2016). ArticleCASGoogle Scholar
- Wilmer, C. E. et al. Large-scale screening of hypothetical metal–organic frameworks. Nat. Chem.4, 83–89 (2012). ArticleCASGoogle Scholar
- Evans, J. D., Jelfs, K. E., Day, G. M. & Doonan, C. J. Application of computational methods to the design and characterisation of porous molecular materials. Chem. Soc. Rev.46, 3286–3301 (2017). ArticleCASGoogle Scholar
- Greenaway, R. et al. High-throughput discovery of organic cages and catenanes using computational screening fused with robotic synthesis. Nat. Commun.9, 2849 (2018). ArticleCASGoogle Scholar
- Pulido, A. et al. Functional materials discovery using energy–structure–function maps. Nature543, 657–664 (2017). ArticleCASGoogle Scholar
- Eliasova, P. et al. The ADOR mechanism for the synthesis of new zeolites. Chem. Soc. Rev.44, 7177–7206 (2015). ArticleCASGoogle Scholar
- Mastalerz, M. & Oppel, I. M. Rational construction of an extrinsic porous molecular crystal with an extraordinary high specific surface area. Angew. Chem. Int. Ed.51, 5252–5255 (2012). ArticleCASGoogle Scholar
- Ferlat, G. et al. van der Waals forces stabilize low-energy polymorphism in B2O3: implications for the crystallization anomaly. Phys. Rev. Mater.3, 063603 (2019). ArticleCASGoogle Scholar
- Zhang, W., Mazzarello, R., Wuttig, M. & Ma, E. Designing crystallization in phase-change materials for universal memory and neuro-inspired computing. Nat. Rev. Mater.4, 150–168 (2019). ArticleCASGoogle Scholar
- Tominaka, S. et al. Topochemical conversion of a dense metal–organic framework from a crystalline insulator to an amorphous semiconductor. Chem. Sci.6, 1465–1473 (2015). ArticleCASGoogle Scholar
- MacFarlane, D. R. et al. Energy applications of ionic liquids. Energy Environ. Sci.7, 232–250 (2014). ArticleCASGoogle Scholar
- Chen, X., Gao, H., Tang, Z. & Wang, G. Metal–organic framework-based phase change materials for thermal energy storage. Cell Rep. Phys. Sci.1, 100218 (2020). ArticleCASGoogle Scholar
- McGillicuddy, R. D., Thapa, S., Wenny, M. B., Gonzalez, M. I. & Mason, J. A. Metal–organic phase-change materials for thermal energy storage. J. Am. Chem. Soc.142, 19170–19180 (2020). ArticleCASGoogle Scholar
- Seo, S. et al. Phase-change ionic liquids for postcombustion CO2 capture. Energy Fuels28, 5968–5977 (2014). ArticleCASGoogle Scholar
- Tozawa, T. et al. Porous organic cages. Nat. Mater.8, 973–978 (2009). ArticleCASGoogle Scholar
- Giri, N. et al. Liquids with permanent porosity. Nature527, 216–220 (2015). ArticleCASGoogle Scholar
- Kinoshita, Y., Matsubara, I., Higuchi, T. & Saito, Y. The crystal structure of bis(adiponitrilo)copper( i ) nitrate. Bull. Chem. Soc. Jpn32, 1221–1226 (1959). ArticleCASGoogle Scholar
- Hoskins, B. F. & Robson, R. Design and construction of a new class of scaffolding-like materials comprising infinite polymeric frameworks of 3D-linked molecular rods. A reappraisal of the Zn(CN)2 and Cd(CN)2 structures and the synthesis and structure of the diamond-related frameworks [N(CH3)4][Cu1Zn11(CN)4] and Cul[4,4′,4′′,4′′′-tetracyanotetraphenylmethane]BF4·xC6H5NO2. J. Am. Chem. Soc.112, 1546–1554 (1990). ArticleCASGoogle Scholar
- Yaghi, O. M., Li, G. M. & Li, H. L. Selective binding and removal of guests in a microporous metal–organic framework. Nature378, 703–706 (1995). ArticleCASGoogle Scholar
- Kondo, M., Yoshitomi, T., Seki, K., Matsuzaka, H. & Kitagawa, S. Three-dimensional framework with channeling cavities for small molecules: <[M2(4,4′-bpy)3(NO3)4]·xH2O>n (M=Co, Ni, Zn). Angew. Chem. Int. Ed.36, 1725–1727 (1997). ArticleCASGoogle Scholar
- Kistler, S. S. Coherent expanded aerogels and jellies. Nature127, 741–741 (1931). ArticleCASGoogle Scholar
- Bergaya, F. & Lagaly, G. Handbook of Clay Science (Elsevier, 2006).
- Stoeckli, H. F. Microporous carbons and their characterization—the present state-of-the-art. Carbon28, 1–6 (1990). ArticleCASGoogle Scholar
- Song, Q. L. et al. Controlled thermal oxidative crosslinking of polymers of intrinsic microporosity towards tunable molecular sieve membranes. Nat. Commun.5, 4813 (2014). ArticleCASGoogle Scholar
- Izatt, R. M. Macrocyclic and Supramolecular Chemistry (Wiley, 2016).
- Rosi, N. L. et al. Hydrogen storage in microporous metal–organic frameworks. Science300, 1127–1129 (2003). ArticleCASGoogle Scholar
- Comas-Vives, A. Amorphous SiO2 surface models: energetics of the dehydroxylation process, strain, ab initio atomistic thermodynamics and IR spectroscopic signatures. Phys. Chem. Chem. Phys.18, 7475–7482 (2016). ArticleCASGoogle Scholar
- Ranganathan, R. et al. Modeling high-temperature diffusion of gases in micro and mesoporous amorphous carbon. J. Chem. Phys.143, 084701 (2015). ArticleCASGoogle Scholar
- Deng, Z. et al. Facilitate gas transport through metal–organic polyhedra constructed porous liquid membrane. Small16, 1907016 (2020). ArticleCASGoogle Scholar
- Debenedetti, P. G. & Stillinger, F. H. Supercooled liquids and the glass transition. Nature410, 259–267 (2001). ArticleCASGoogle Scholar
- Widmer, R. N. et al. Pressure promoted low-temperature melting of metal–organic frameworks. Nat. Mater.18, 370–376 (2019). ArticleCASGoogle Scholar
- Ueda, T., Tominaga, T., Mochida, T., Takahashi, K. & Kimura, S. Photogeneration of microporous amorphous coordination polymers from organometallic ionic liquids. Chem. Eur. J.24, 9490–9493 (2018). ArticleCASGoogle Scholar
Acknowledgements
T.D.B. acknowledges the Royal Society for a University Research Fellowship (UF150021), the Leverhulme Trust for a Philip Leverhulme Prize and the University of Canterbury Te Whare Wānanga o Waitaha, New Zealand, for a University of Cambridge Visiting Canterbury Fellowship. F.-X.C. acknowledges funding by the Agence Nationale de la Recherche (ANR-18-CE29-0009-01). A.I.C. acknowledges the Leverhulme Trust for funding through the Leverhulme Research Centre for Functional Materials Design.
Author information
Authors and Affiliations
- Department of Materials Science and Metallurgy, University of Cambridge, Cambridge, UK Thomas D. Bennett
- Chimie ParisTech, PSL University, CNRS, Institut de Recherche de Chimie Paris, Paris, France François-Xavier Coudert
- School of Chemistry and Chemical Engineering, Queen’s University Belfast, Belfast, UK Stuart L. James
- Materials Innovation Factory and Department of Chemistry, University of Liverpool, Liverpool, UK Andrew I. Cooper
- Leverhulme Research Centre for Functional Materials Design, Materials Innovation Factory and Department of Chemistry, University of Liverpool, Liverpool, UK Andrew I. Cooper
- Thomas D. Bennett